Asian Monsoon and Troposphere-Stratosphere Coupling
1. The Asian Monsoon and the Structure of the Lower and Upper Troposphere
Subtropical High-Pressure Zone
The circulation of the atmosphere is a kind of heat engines. Regional differences in heating by solar radiation cause high and low temperature areas that result in atmospheric motion along with the flow of heat from the warm area(s) to the cold area(s).
The mechanism that converts the temperature gradient into work occurs through the thermal expansion in the atmosphere (Charles's law) and gravity. In addition, due to stratification of the atmosphere and the Coriolis force, flow is maintained as a large-scale horizontal flow without cascading into fine, three-dimensional, turbulent flows.
Since the temperature gradient is large in the latitudinal direction, zonal wind is dominant due to thermal wind balance. Furthermore, because of the presence of water, latent heat release associated with tropical convection drives the tropospheric circulation as an additional heat source. This heat source forms a tropical meridional circulation (Hadley cell) due to the nonlinear balance and maintains a strong subtropical jet that separates the low-latitude atmosphere from the mid and high-latitude atmosphere (Held and Hou, 1980).
Typically, the tropics experience upwelling and low pressure accompanied by the formation of pronounced cumulonimbus clouds, while the subtropics experience downwelling and high pressure. This causes the subtropical high-pressure zone and the dry and hot Sahara and other deserts in the subtropics.
Zonally-Asymmetric Circulation in the Subtropics
Actually, the pressure pattern in the subtropics is essentially zonally non-uniform. In other words, the surface pressure is not a single high-pressure zone but a series of stationary highs and lows in the zonal direction. This tendency is more pronounced in summer in both hemispheres.
This is inconsistent with the upwelling and low-pressure areas in the summer hemisphere and downwelling and high-pressure areas in the winter hemisphere (Linzen and Hou, 1988), which are expected in axisymmetric models (Rodwell and Hoskins, 1996; Miyasaka and Nakamura, 2005, as mentioned in the intro). Hence, actual subtropical anticyclones cannot be adequately explained by these models, and it is more appropriate to consider them as being formed by zonally non-uniform dynamics.
The climatological pressure pattern in the subtropics is shown in Figs. 1 and 2 for the surface and near the tropopause.
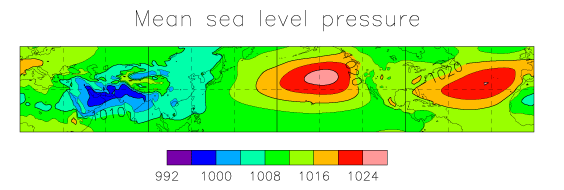
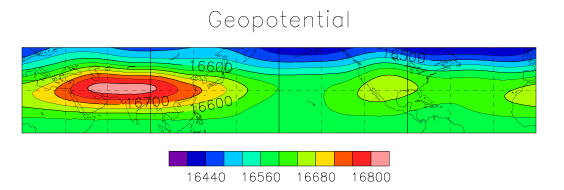
The characteristics of the monthly/seasonally averaged subtropical circulation in the boreal summer are summarized below.
-
In terms of sea-level pressure, there are two high pressure systems over the ocean and two low pressure systems over the continent.
The two anticyclones are centered over the eastern Pacific and Atlantic Oceans and are called the Ogasawara High and Azores High, respectively. -
The geopotential heights from the upper troposphere to the tropopause (at 200 hPa and 100 hPa) are almost opposite those in the lower troposphere given above.
There is a trough over the Pacific and eastern Atlantic, and an anticyclone over Eurasia and North America. The anticyclone over Eurasia is particularly strong and large enough to reach from 0° to 30° E. In the latitudinal direction, it reaches its maximum at approximately 30° N.
There are three main types of thermal forcing that form this circulation, as follows: (i) the sensible heat flux from the surface that reflects the land–sea distribution, (ii) the latent heat release associated with convection, and (iii) longwave radiation in the atmosphere. Interpretation is not straightforward because each has its own feedback processes with clouds. Many numerical studies have performed to determine the main factor(s) causing the zonally asymmetric circulation. Presently, the dominant view is that the sensible heat flux in the Pacific and Atlantic highs in the lower troposphere (Miyasaka and Nakamura, 2005) and latent heat release by convective clouds in the upper troposphere (Rodwell and Hoskins, 2001; Chen et al., 2001) each play major roles.
Asian Monsoon
The low-pressure regions over Asia and North America, shown in Fig. 1, each have cyclonic circulation that blows northward toward the continent. This is the (summer) monsoon, and the Asian monsoon is well known as the largest of its kinds globally. The horizontal winds in the lower troposphere (850 hPa) and upper troposphere (100 hPa) in July near Asia are shown in Figs. 3 and 4, along with precipitation and outgoing longwave radiation (OLR).
Alongside the lower tropospheric circulation, there is a strong convergence of winds, especially around the Bay of Bengal, as well as locally large amounts of precipitation. The latent heat release associated with the high precipitation acts as a large heat source, which, in turn, drives the circulation there.
In the upper troposphere and lower stratosphere, a large anticyclone, known as the Asian summer monsoon anticyclone, covers the region from West to Southeast Asia. It is occasionally also called the Tibetan anticyclone or the South Asian High.
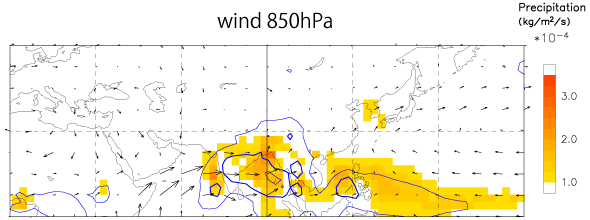
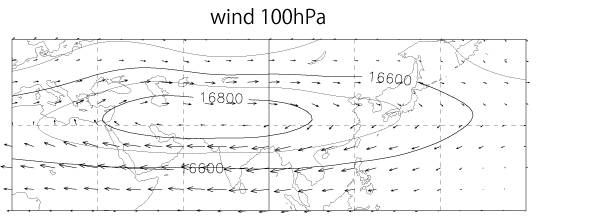
Large-scale upwelling, driven by the thermal forcing associated with convection near the Bay of Bengal, results in a steady horizontal wind divergence in the upper troposphere.
Thus, from the perspective of linear dynamics, the Asian monsoon anticyclone is considered to be a steady Rossby-wave response forced by horizontal wind divergence in the upper troposphere.
However, because this thermal forcing is remarkably large, understanding the actual structure of the anticyclone and the complex behavior of its intra-seasonal variability requires a focus on the nonlinearity of its dynamics.
2. Roles in Material Transport and Mixing
Although the importance of the summer Asian monsoon to the climate has long been recognized, it was only recently that the circulation near the tropopause received attention, with Dunkerton (1995) being the first to suggest its importance based on observational data. Additionally, Chen (1995), using a semi-Lagrangian transport model, showed that the anticyclonic circulation, especially the meridional flow, contributes significantly to the mass exchange between the stratosphere and troposphere on isentropic surfaces above 340 K.
The singularities of the atmospheric structure near the tropopause associated with the Asian monsoon anticyclone can be schematically represented as follows.
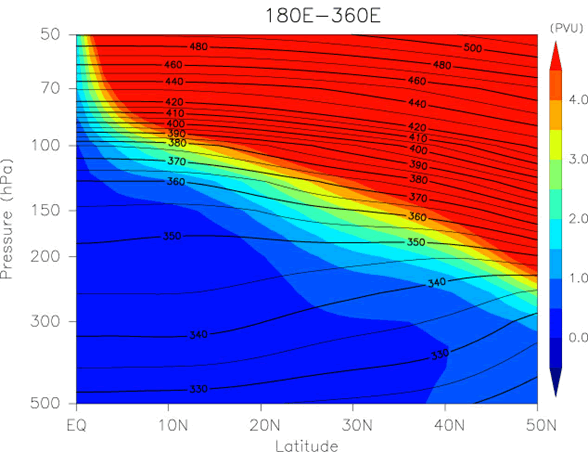
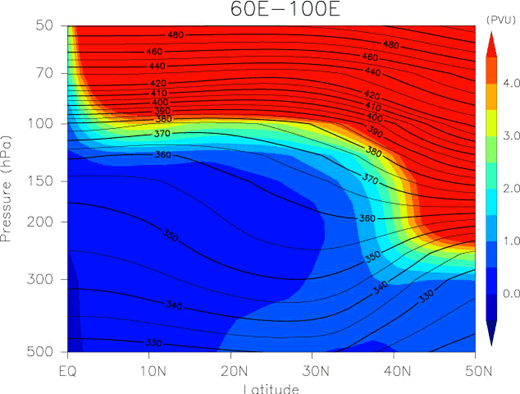
As shown in Figs. 5 and 6 above, the altitude of the tropopause rises in the vicinity of the Asian monsoon anticyclone due to diabatic heating caused by the strong convection. On the isobaric and isentropic surfaces near the tropopause, the inside of the anticyclone belongs to the troposphere and the outside belongs to the stratosphere.
As a result, the mixing ratios of chemical substances differs greatly inside and outside of the anticyclone. In recent years, satellite observations have provided high-resolution data on various atmospheric trace elements that support this theory. For example, Figs. 7 and 8 below show the distributions of the mixing ratio of carbon monoxide and ozone at approximately 100 hPa, as observed by the Aura microwave limb sounder (MLS) (Park et al., 2007). The mixing ratio of carbon monoxide is high inside the monsoon anticyclone, while that of ozone is low. Thus, a large fraction of the air is derived from the troposphere.
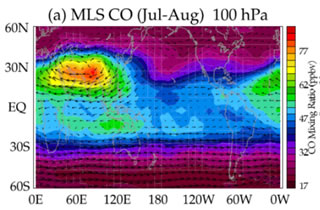
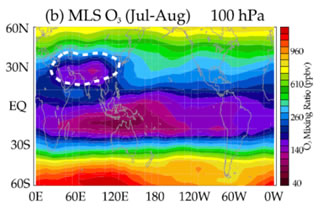
Potential Vorticity (PV) as a Tracer
Considering the large differences in the chemical properties between the air inside and outside of the Asian monsoon anticyclone, as described above, irreversible mixing associated with the horizontal flow is important. To discuss such mass mixing, diagnostic analysis using potential vorticity (PV) on an isentropic (constant potential temperature) surface is very useful.
Both potential temperature and PV are conserved quantities under adiabatic and non-dissipative conditions. Thus, PV can be used as a passive tracer over a few days. The PV in the stratosphere is significantly larger than that in the troposphere. The PV distribution provides significant insights into irreversible mixing of material inside and outside the vortex.
Although processes occurring in the region near the tropopause are not necessarily adiabatic due to the influence of convective clouds and longwave radiation, PV on an isentropic surface specifically in the region of 360–400 K is sufficiently close to a conserved quantity and its distribution corresponds well to the observed distribution of chemical species.
Comparison to the Polar Vortex
The Asian monsoon anticyclone and polar vortex are both planetary-scale isolated vortices. The polar vortex, which develops in the polar stratosphere from winter to spring, has been an important topic for research on mass transport and mixing, especially in relation to the ozone hole problem (Fig. 10). Many of the methods developed for studying the polar vortex can also be applied to the Asian monsoon anticyclone.
The structure of both vortices is approximately barotropic, and their two-dimensional structure can only be determined from the PV on isentropic surfaces. The PV has a large gradient at the edge of the vortices, indicating that irreversible mixing is suppressed. In the case of the polar vortex, methods to diagnose the material mixing based on the PV conservation have been developed. These methods have been applied to the Asian monsoon anticyclone. For example, the area surrounded by a PV contour is used as an indicator of vortex strength (Garny and Randel, 2013). The PV gradient in the direction of equivalent latitude, which is defined based on PV distribution, is used to diagnose the boundary between the inside and outside of the vortex (Ploeger et al., 2015).
Unlike the polar vortex, the Asian monsoon anticyclone is not symmetric, but flatter in the zonal direction (see the region of low ozone mixing ratio and high geopotential height in Fig. 9). In addition, because it is located at low latitudes, it borders the subtropical jet on the north side, whereas its southern side exists in the tropics, where the Coriolis force is weak. Hence, it is difficult to perform operations such as zonal averaging (circumferential averaging), which is effective for the polar vortex. Because the dynamics of the Asian monsoon anticyclone are essentially different from those of the polar vortex, a new method for understanding transport and mixing is needed.
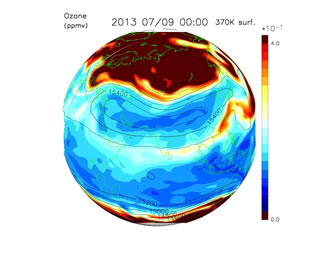
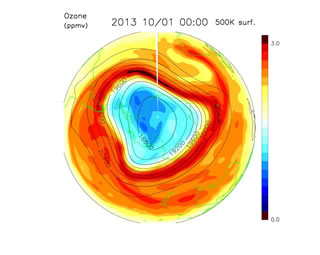
3. Intra-Seasonal Variation
The Asian monsoon is characterized by well-known, pronounced intra-seasonal variability in the wind, pressure, and precipitation in the troposphere (e.g., Krishnamurti, 1973), with a predominant period of about two weeks or one month. The Asian monsoon anticyclone near the tropopause also shows active intra-seasonal variability in both intensity and shape.
Variations in the shape of the monsoon anticyclone are most conveniently captured by PV, which can be regarded as a conserved quantity. During the summer, the low PV area corresponding to the anticyclone shows active daily fluctuation, as shown in Fig. 11 below. The distribution of the mixing ratios of troposphere-derived species trapped in the anticyclone shows variations similar to those of the PV distribution.
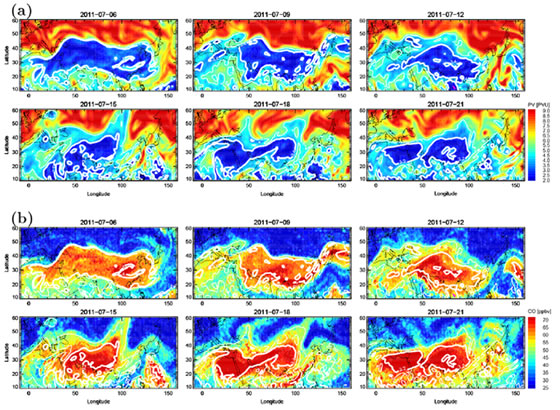
Associated with these variations, the anticyclone center, determined by a geopotential maximum, etc., also shifts significantly in the zonal direction, coming and going between two positions. For example, Fig. 12 shows the frequency distribution of the center longitude of the anticyclone during the summer (Zhang et al., 2002), which was detected by using geopotential heights at 100 hPa. The center position, rather than smoothly transitioning between east and west, intermittently transitions between one of the two eastern (Tibetan mode) and western (Iranian mode) centered states (Fig. 13; Zhang et al., 2002). Thus, the intra-seasonal variability of the Asian monsoon anticyclone has a "bimodal" characteristic.
This variability is also known as “quasi-biweekly oscillation”, having a predominant period of approximately two weeks.
The relationship between such fluctuations near the tropopause and the pressure and precipitation variations in the troposphere is another recent research topic (Ortega et al., 2017).
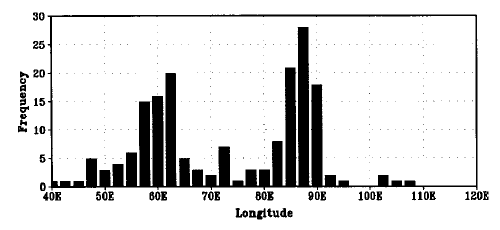
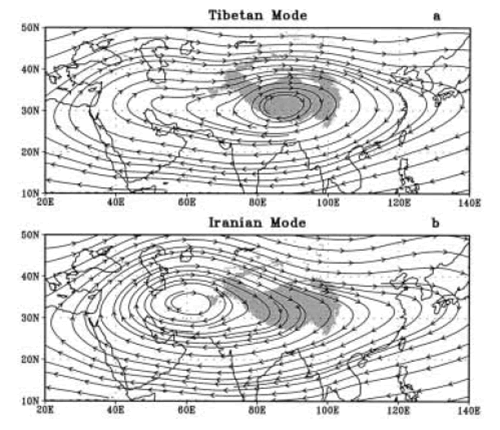
Causes of Intra-Seasonal Variation
Along with externally forced variability, such as diabatic heating due to convection, internal variability may also be involved in the anticyclonic variability. The monsoon anticyclone itself is unstable due to large-scale balanced flows because the latitudinal gradient of the potential vorticity is negative on the low latitude side. Nevertheless, it is uncertain which of these factors actually determines the variability of the anticyclone.
The Asian monsoon anticyclone is primarily formed and maintained by diabatic heating due to convective activity associated with the tropical and subtropical monsoons (especially around the Bay of Bengal). The convective activity itself shows active intra-seasonal variability. Thus, it is naturally inferred that the anticyclone variability is a response to the variability in convective activity. Garny and Randel (2013) defined anticyclone strength in terms of the area enclosed by a PV contour on a isentropic surface and correlated it with the variabilities of convection and upper tropospheric divergence, which is excited by convection. Several studies have also shown lag correlations between active convection and the strength of the anticyclone above and to the west of the convection.
On the other hand, by focusing on the dynamic instability of the Asian monsoon anticyclone, the intra-seasonal variability can be explained as an internal property of the anticyclone itself. Hsu and Plumb (2000) and Lin et al. (2007) used a shallow-water system and a mechanistic model, respectively, to show that periodic variability of the anticyclone occurs under steady thermal forcing.
Nevertheless, our understanding, based on relatively simple models, is limited. Further, dynamical and thermodynamical roles of the Tibetan Plateau and influences of the jet are also issues for future research.
A quantitative understanding that integrates these different factors is important for elucidating the behavior of the Asian monsoon anticyclone and its future intra- and inter-annual variabilities.
(Japanese version written by Arata Amemiya, March 2017)
- General Circulation in the Middle Atmosphere
- Generation, Propagation, and Spectra of Atmospheric Gravity Waves
- Stratospheric sudden warming and elevated stratopause events
- Dynamics in the Mesosphere: Interplay of Rossby Waves and Gravity Waves
- International collaborative study of interhemispheric coupling by a global network of mesosphere-stratosphere-troposphere radars
- Program of the Antarctic Syowa MST/IS radar (PANSY)
- Gravity-wave permitting high-resolution middle atmosphere general circulation model studies (KANTO)
- Asian Monsoon and Troposphere-Stratosphere Coupling